Method for evaluating solar energy absorption capability of regional electric-thermal integrated energy system
A technology that integrates energy systems and absorbing capacity, and is used in heating systems, hot water central heating systems, heating methods, etc.
- Summary
- Abstract
- Description
- Claims
- Application Information
AI Technical Summary
Problems solved by technology
Method used
Image
Examples
specific Embodiment
[0253] In particular, as figure 2 In this embodiment, a regional electric-thermal integrated energy system composed of an IEEE33 regional power system and a 51-node regional thermal system is selected, and its topology is as follows figure 2 shown. The system contains two energy stations. The equipment in each energy station includes Photovoltaic (PV), Solar Collector (SC), Gas Turbine Combined Heat and Power (CHP) unit, Electric Boiler (Electrical) boiler, EB). In this example, three typical days of winter (120 days, determined according to the heating period), transition season (153 days), and summer (92 days) are used to verify the solar energy absorption capacity evaluation model.
[0254] In this embodiment, four sets of calculation examples are selected to verify the solar energy absorption capacity evaluation model of the regional electric-thermal integrated energy system proposed in the present invention, to explore the optimal solar energy configuration and operat...
PUM
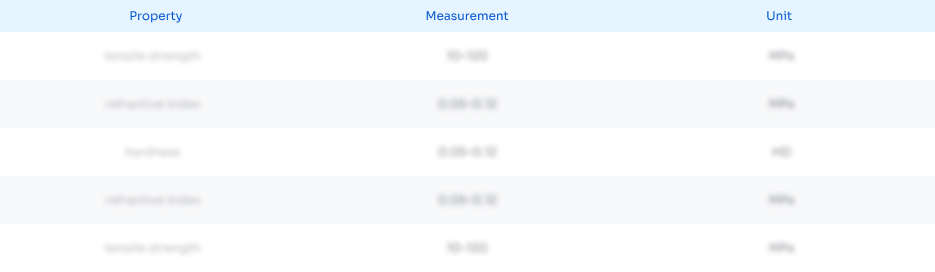
Abstract
Description
Claims
Application Information

- R&D
- Intellectual Property
- Life Sciences
- Materials
- Tech Scout
- Unparalleled Data Quality
- Higher Quality Content
- 60% Fewer Hallucinations
Browse by: Latest US Patents, China's latest patents, Technical Efficacy Thesaurus, Application Domain, Technology Topic, Popular Technical Reports.
© 2025 PatSnap. All rights reserved.Legal|Privacy policy|Modern Slavery Act Transparency Statement|Sitemap|About US| Contact US: help@patsnap.com