Method for controlling dynamic direct voltage of parallel connection type active electric filter
A power filter, DC voltage technology, applied in active power filter, harmonic reduction device, AC network to reduce harmonics/ripple, etc., can solve unsolved problems such as DC voltage control, to improve dynamic performance, The effect of improving performance
- Summary
- Abstract
- Description
- Claims
- Application Information
AI Technical Summary
Problems solved by technology
Method used
Image
Examples
Embodiment
[0049] Embodiment: A method for controlling dynamic DC voltage of a parallel active power filter, characterized in that:
[0050] Step 1, the charging current from the grid precharges the DC side capacitor through the contactor, current limiting resistor, grid side inductance, converter side inductance, and uncontrolled rectifier bridge; this step 1 further includes the following steps: Step 1-1 1. The pre-charging contactor KM2 is closed and the main contactor KM1 is disconnected. Under the action of the grid voltage, the charging current passes through the pre-charging contactor KM2, current limiting resistor, grid-side inductance, converter-side inductance, and uncontrolled rectifier bridge. , and finally flows into the DC capacitor, the DC voltage continues to rise until the charging current is close to zero, and the DC voltage reaches a stable value Udc1; step 1-2, after the charging is stable, the main contactor KM1 is closed, the current limiting resistor Rs and the pre-...
PUM
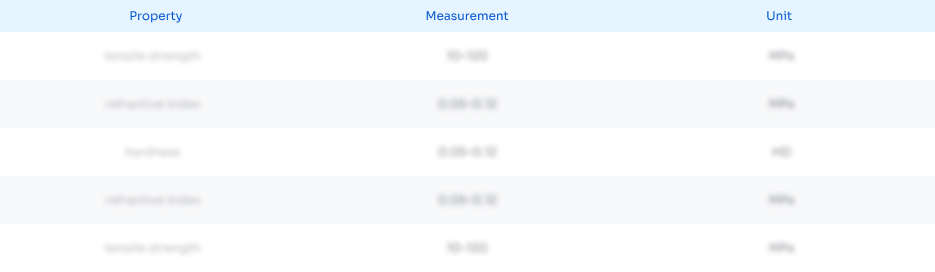
Abstract
Description
Claims
Application Information

- R&D
- Intellectual Property
- Life Sciences
- Materials
- Tech Scout
- Unparalleled Data Quality
- Higher Quality Content
- 60% Fewer Hallucinations
Browse by: Latest US Patents, China's latest patents, Technical Efficacy Thesaurus, Application Domain, Technology Topic, Popular Technical Reports.
© 2025 PatSnap. All rights reserved.Legal|Privacy policy|Modern Slavery Act Transparency Statement|Sitemap|About US| Contact US: help@patsnap.com